Successfully applying regenerative medicine in the clinic depends on precisely characterising stem cell populations, including assessing function in electrically active cells, which is possible using microelectrode arrays
The field of regenerative medicine continues along its trajectory to transform healthcare. Scientists and physicians hope that cell transplantation therapies made from induced pluripotent stem cells (iPSCs) can one day replace injured or diseased tissue for many conditions including amyotrophic lateral sclerosis, heart failure, Type 1 diabetes, and spinal cord injury. Confirming that differentiated iPSCs will perform as intended has historically been challenging, but bioelectronic assays provide a promising platform for testing the functionality of electrically active cells such as neurons and cardiomyocytes.
The discovery of iPSCs was a breakthrough for regenerative medicine. First, scientists learned that cell plasticity is not as restricted as once believed. Second, they found that any cell can be reprogrammed back to an immature state and then influenced to become another cell type. This discovery effectively eliminated ethical concerns related to obtaining iPSCs from embryonic tissue. However, scientists today still face the challenge of developing cell types in vitro that behave as they would in the body. This challenge makes it critical that scientists characterise differentiated iPSCs as fully as possible using various assays to confirm their identity and functionality.
Examining Differentiated iPSC Populations
Following the discovery of iPSCs in 2006, researchers have drastically expanded and improved protocols for differentiating them. It is now possible to induce specific cell subtypes or promote the maturity of the differentiated cell population. For example, iPSC-derived cardiomyocytes can be induced to become more ventricular, atrial, or nodal-like in their composition, each having a unique profile (1). Similarly, scientists have also described cell type-specific differentiation methods for neurons to produce a rather impressive list of cell types generated from iPSCs: motor, dopaminergic, GABAergic, cortical, and pyramidal, to name a few.
Despite their sophistication, existing differentiation protocols are imperfect – none achieve 100% efficiency, and scientists need to perform additional steps to obtain a purified population of cells. The level of differentiation is also of particular significance to regenerative medicine, as cells that are too immature might not appropriately mimic human cells, and may increase one’s cancer risk if they receive these cells as treatment (2). It is paramount that scientists precisely characterise the cells they produce from iPSCs, especially considering the sensitivity of the differentiation process, the heterogeneity of the resulting cell population, and the importance of mature phenotypes (2, 3).
Comparing iPSC Characterisation Tools and Methodologies
There are many methods for characterising the differentiated product of iPSCs. Often, creating a molecular and functional profile involves confirming the cells’ morphology and appropriate genetic or protein expression, as well as evaluating any unique function of a given cell type for an appropriate response to stimuli. For electrically active cell populations, such as cardiomyocytes and neurons, this process can be more complex due to the cells’ electrophysiological properties. These properties can vary based on maturity and cell subtype and can be used to gauge the fitness of the cell.
The most traditional method available to interrogate the electrophysiology of cells is patch-clamp recording.
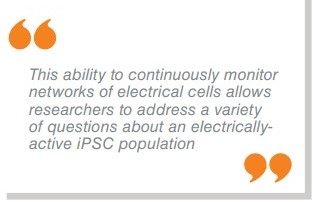
Despite the value of this technique in basic research, it is not practical for the functional assessment of many iPSC populations. Patch-clamp requires significant training and skill. It is a time-consuming process that can only measure the electrical behaviour of one cell, or measure the interaction between two cells, at a time.
Alternatively, bioelectronic assays, based on microelectrode array (MEA) technology, provide a simple, label-free setup that enables scientists to measure whole networks of cells non-invasively and over time, without the need for specialised training. This makes it possible to study electrically active cells as they mature and develop connections with the cells around them. While there are a few types of MEAs, multiwell MEA platforms are well suited for this purpose. They consist of a cell culture plate equipped with a grid of tightly spaced, highly sensitive microelectrodes embedded in the bottom of each well; the electrodes do not interfere with the cells. The cells are then plated in the wells over the electrodes. Each embedded electrode captures spontaneous or evoked electrical activity over the desired timeframe in real time.
Applications of MEA Technology
This ability to continuously monitor networks of electrical cells allows researchers to address a variety of questions about an electrically-active iPSC population. As mentioned previously, maturity and cell subtype are major considerations in regenerative medicine. In the case of cardiomyocytes, fetal-like and adult-like cells exhibit unique electrophysiological properties due to the differences in ion channel densities (1). MEAs can measure action potential parameters of iPSC cardiomyocytes to assess their maturation status. Cardiomyocyte cell subtypes also have distinct electrical waveforms that are discernable on an MEA, with the ventricular type having a pronounced plateau phase and longer duration than the atrial subtype (see Figure 1).
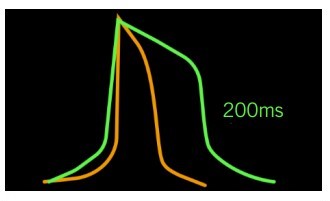
Figure 1: Schematic demonstrating the unique action potential profiles between cardiomyocyte subtypes. An atrial cardiomyocyte action potential is short with a minimal plateau phase (orange) while a ventricular cardiomyocyte action potential is longer and has a prominent plateau phase (green)
In the case of neural networks, MEAs can help scientists discern between many distinct cell subtypes and capture their complex connectivity (4). MEA recordings can yield data regarding mean firing rate, synchrony index, burst rate, burst duration, burst intensity, number of spikes, and other metrics that provide insight into unique cell-specific electrophysiological qualities of neuronal cells (4, 5). As they can describe neurons using multiple parameters, scientists can be highly specific in their characterisation of these cells. Importantly, these data include timing and spatial information, further helping to describe neural networks. Since each cell type has its own identifying electrical activity pattern, the assay can deduce whether the differentiation protocol yielded the appropriate cell type. These distinct activity patterns reflect the receptors and activated cell signalling pathways that define a cell and dictate its function within the body. Therefore, this functional measure complements the molecular characterisation of the cell.
Scientists know that the behaviour of an isolated cell population studied in vitro is not fully representative of their behaviour in vivo, where the cells reside near and interact with multiple cell types. Indeed, this microenvironment is critical for neuronal cells, as their ability to form proper connections with other neurons depends on supporting glial cells such as astrocytes (5). A key benefit of MEAs is that they can support co-culture systems to better model the expected behaviour of the differentiated cell in an appropriate environment (see Figure 2). It is also possible to compartmentalise two neuronal networks to observe their ability to establish a connected circuit, which is another functional consideration for neuronal iPSCs (6).
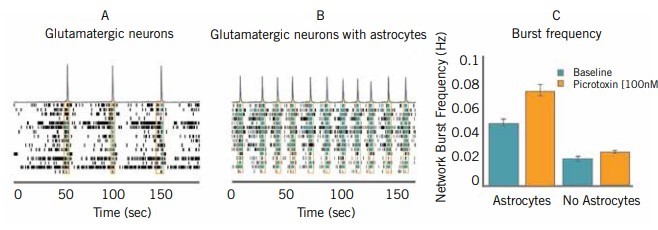
Figure 2: Raster plots generated from MEA measurements of iPSC-derived neurons without (A) and with (B) astrocytes. Neural-glial interactions are important regulators of activity in health and disease. Their impact on neuronal function can be modeled on the MEA (C)
Scientists are also interested in using electrical stimulation to develop neuronal network function and plasticity of iPSC cells, thereby achieving a more highly developed population containing more differentiated and mature cells (5, 7, 8). Since the electrodes embedded in the wells of the MEA are also able to stimulate electrical activity, this is another potential and valuable use of bioelectronic assays (9).
Driving Understanding of Diseases and Biological Mechanisms
While the success of regenerative medicine is contingent upon the quality of the cells intended for transplantation, therapeutic developers also need an adequate understanding of the disease they are attempting to treat (2). Fortunately, bioelectronic assays can help contribute to our knowledge of injuries and degenerative diseases. Researchers can perform reprogramming and differentiation protocols on cells collected from patients with a specific disease (10). These patient-derived iPSCs can be studied using MEA technology to identify unique electrophysiological profiles of the disease and assess the ability of new treatments to normalise aberrant electrical activity. This strategy is employed widely for many diseases, and has contributed considerably to the field of regenerative medicine (5).
MEA technology has a multitude of capabilities that enhance the study of stem cells, thereby advancing the field of regenerative medicine. The bioelectronic assay offers a simple, non-invasive apparatus for the analysis of electrophysiological parameters that is widely accessible to researchers from any area of molecular biology. As the field works towards refining iPSC protocols and subsequent characterisation of differentiated cell types, scientists will start to see the impact of the cells in the clinic as a new and exciting branch of medicine.
References
- Yoshida Y et al, Induced Pluripotent Stem Cells 10 Years Later: For Cardiac Applications, Circulation Research, pp1,958-1,968, 2017.
- Rehakova D et al, Clinical-Grade Human Pluripotent Stem Cells for Cell Therapy: Characterization Strategy, International Journal of Molecular Sciences, 2020.
- Eguizabal C et al, Two decades of embryonic stem cells: a historical overview, Human Reproduction Open, 2018.
- Mossink B et al, Human neuronal networks on microelectrode arrays are a highly robust tool to study diseasespecific genotype-phenotype correlations in vitro, Stem Cell Reports, pp2,182-2,196, 2021.
- McCready FP et al, Multielectrode Arrays for Functional Phenotyping of Neurons from Induced Pluripotent Stem Cell Models of Neurodevelopmental Disorders, Biology, 2022.
- Millard D et al, Characterization of an in vitro synaptic propagation assay using iPSC-derived neurons and multiwell microelectrode array technology, Axion Biosystems Poster, ISSCR 2021 Poster number 777.
- Pelkonen A et al, Functional Characterization of Human Pluripotent Stem Cell-Derived Models of the Brain with Microelectrode Arrays, Cells, 2022.
- Napoli A et al, Investigating brain functional evolution and plasticity using microelectrode array technology, Brain Research Bulletin, pp127-135, 2015.
- Latchoumane CFV et al, Chronic Electrical Stimulation Promotes the Excitability and Plasticity of ESC-derived Neurons following Glutamate-induced Inhibition In vitro, Scientific Reports, 2018.
- Aboul-Soud MAM et al, Induced Pluripotent Stem Cells (iPSCs) – Roles in Regenerative Therapies, Disease Modelling and Drug Screening, Cells, 2021.